Direct Fabrication: Overview
Producing Metal Parts with Selective Laser Sintering/Hot Isostatic Pressing
Suman Das, Martin Wohlert, Joseph J. Beaman, and David L. Bourell
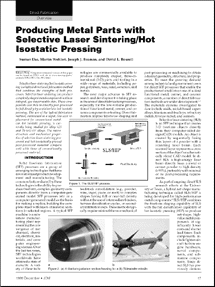 |
CONTENTS |
---|
|
Selective laser sintering/hot isostatic pressing is a hybrid direct laser fabrication method that combines the strengths of both processes. Selective laser sintering can produce complexly shaped metal components with an integral, gas-impermeable skin. These components can then be directly post-processed to full density by containerless hot isostatic pressing. The use of the hybrid fabrication method, envisioned as a rapid, low-cost replacement for conventional metal-can hot isostatic pressing, is currently being studied for alloy 625 and Ti-6Al-4V alloys. The microstructure and mechanical properties of selective-laser-sintering processed and hot isostatically pressed post-processed material compare well with those of conventionally processed material.
Solid freeform fabrication (SFF) processes are a group of emerging technologies that have revolutionized product development and manufacturing. The common feature shared by these technologies is the ability to produce freeform, complex-geometry components directly from a computer-generated model. SFF processes rely on a computer-generated model as the basis for making a replica, building the complete object with layers of material additions in selected regions. A typical SFF machine is a miniature manufacturing plant representing the convergence of mechanical, chemical, electrical, materials, and computer engineering sciences. Over the last ten years, SFF technologies worldwide have attained a state of maturity. A variety of these technologies are commercially available to produce complexly shaped, three-dimensional (3-D) parts and tooling in a wide range of materials, including paper, polymers, wax, sand, ceramics, and metals.
The next major advance in SFF research and development is taking place in the area of direct fabrication processes, especially for the low-volume production of functional metal, cermet, and ceramic components or tooling. Direct fabrication implies layerwise shaping and feedstock consolidation (e.g., powder, wire, ingot, paste, or melt) to complex shapes having full or near-full density without the use of intermediate binders, furnace densification cycles, or secondary infiltration steps. These methods typically require minimal thermomechanical post-processing or machining to obtain a desired geometry, structure, and properties. To meet the growing demand among industrial and government users for direct SFF processes that enable the production of small lots or one-of-a-kind functional metal, cermet, and ceramic components, a number of direct fabrication methods are under development.1-7 The materials systems investigated to date include steels, nickel-based superalloys, titanium and its alloys, refractory metals, bronze-nickel, and cermets.
Selective laser sintering (SLS) is an SFF technique that creates 3-D freeform objects directly from their computer-aided design (CAD) models. An object is created by sequentially fusing thin layers of a powder with a scanning laser beam. Each scanned layer represents a cross section of the object's mathematically sliced CAD model. In direct SLS, a high-energy laser beam directly fuses a metal or cermet powder to high density (>90%), preferably with minimal or no post-processing requirements.
As part of ongoing direct SLS research efforts at the University of Texas, a hybrid net-shape manufacturing technique called SLS/HIP is being developed for high-performance metal components.8 SLS/HIP combines the freeform shaping capability of SLS with the full densification capability of hot isostatic pressing (HIP) to produce net-shape, high-value metal components at significantly lower costs and shorter lead times. Such components include fighter-aircraft turbine engine hardware, naval components, and submarine components. Since direct SLS has previously demonstrated the potential to consolidate metal powders to net shapes with densities in excess of 80 percent theoretical density,9 SLS/HIP is envisioned as a natural combination of SLS and containerless HIP to obtain full-density metal parts.
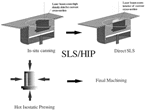 |
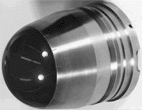 |
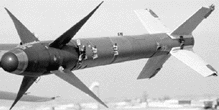 |
Figure 1. The SLS/HIP process. |
Figure 2. (a) A titanium guidance section housing for a (b) Sidewinder missile. |
A schematic of the SLS/HIP process is shown in Figure 1. The working principle of the process is to consolidate the interior of a component to 80 percent or higher density and fabricate an integral gas impermeable skin or "can" at the part boundaries in-situ.10 A component is produced by selectively consolidating a metal powder layer by layer with a laser beam. Unlike other direct laser fabrication processes (e.g., DLF4, LENSTM2, and DMD3) where the objective is to fuse powder to full density across each layer, the laser beam in SLS/HIP fuses the metal powder only at the part boundaries to form an integral gas impermeable skin to a density exceeding 92 percent theoretical density. This is the fractional density at which porosity typically changes from interconnected or surface-connected to closed.11 The powder in the interior of each layer cross section can be optionally laser sintered to an intermediate density typically exceeding 80 percent of theoretical density. Thus, the net-shape powder metallurgy part is shaped, canned, evacuated, and scaled in-situ. The integrally canned, net-shape part produced by SLS is directly post-processed by containerless HIP to full density. A final machining step on critical surfaces may be applied if necessary.
 |
Figure 3. A half-axial section of an SLS-processed alloy 625 cylinder. |
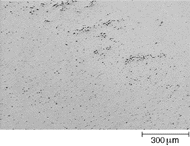 |
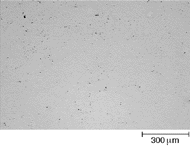 |
Figure 4. Alloy 625 (a) SLS-processed to 98.5 percent density and (b) HIP post-processed to 99.5 percent density. |
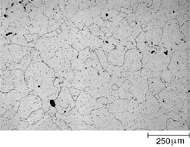 |
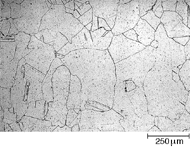 |
Figure 5. The etched microstructure of (a) SLS-processed and (b) SLS/HIP-processed alloy 625. |
SLS/HIP has several advantages over conventional HIP methods. Since an integral skin is formed from the same material as the component, a secondary canning step is not necessary. Adverse container-powder interactions are eliminated, and post-HIP container removal is not required. Tooling and preproc-essing steps associated with container fabrication and filling are also eliminated. Therefore, SLS/HIP enables the production of complex shapes at reduced cost and shorter lead-times.
Under the ongoing research program, SLS/HIP process development is being undertaken for two materials and two demonstration components. Based on a survey8 of several naval installations, the materials that were selected for technology development and demonstration are alloy 625 and Ti-6Al-4V alloys. The component that was selected for SLS/HIP technology demonstration in Ti-6Al-4V is the guidance section housing for the AIM-9 Sidewinder missile (Figure 2).
To demonstrate the feasibility of constructing integrally canned shapes by SLS suitable for HIP post-processing, a number of cylindrical specimens were fabricated by SLS. Two types of cylindrical specimens were produced from alloy 625. In the first type, the cylindrical specimen was 1.27 cm in diameter with 0.31 cm wall thickness and an unprocessed powder core. A half-axial cross-section of such a cylinder is shown in Figure 3. The fractional density varies from 60 percent at the axis to more than 98.5 percent at the diameter. The second type of cylinder was processed to near full density across the entire diameter. This type of processing is necessary to fabricate "end-caps" for an integrally canned object made by SLS suitable for HIP post-processing. Metallographic evaluation revealed the density in this type of specimen to be uniformly in excess of 98.5 percent. Both types of cylinders were post-processed by HIP to full density (99.5 percent of theoretical density). Micrographs of SLS-processed and HIP post-processed alloy 625 are shown in Figure 4.
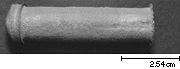 |
Figure 6. A typical SLS-processed integrally canned Ti-6Al-4V cylindrical specimen. |
The etched, microstructure of Inconel 625 processed by SLS (Figure 5a) reveals an equiaxed microstructure. This microstructure compares well with that obtained by hot-rolled annealed material.12 The SLS-processed specimen was post-processed by a HIP cycle of three hours at 1,240°C and 172 MPa. The microstructure of a HIP post-processed SLS specimen is shown in Figure 5b.
For Ti-6Al-4V, integrally canned cylindrical specimens 1.27 cm in diameter and 5 cm long with 0.25 cm skin wall thickness were produced by SLS. A typical integrally canned Ti-6Al-4V cylindrical specimen is shown in Figure 6. These specimens were post-processed to full density by a HIP cycle of four hours at 925°C and 103 MPa. To compare the microstructure with conventionally canned and HIPed Ti-6Al-4V, a reference specimen from the same lot of powder was encapsulated in glass and post-processed using the same HIP cycle. The results, shown in Figure 7, indicate that the microstructure of SLS/HIP-processed Ti-6Al-4V in the bulk is the same as that of conventionally canned and HIPed Ti-6Al-4V.
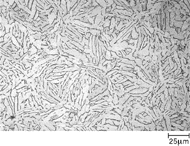 |
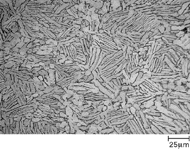 |
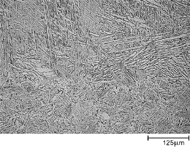 |
Figure 7. The etched microstructure of (a) glass encapsulation HIP Ti-6Al-4V and (b) SLS/HIP-processed Ti-6Al-4V (Kroll's reagent). |
Figure 8. The interface between skin and core in SLS/HIP-processed Ti-6A-4V (Kroll's reagent). |
The skin region of an SLS/HIP-processed Ti-6Al-4V part exhibits a microstructure different from that seen in the bulk. This is expected because the skin region is completely melted and resolidified during SLS and undergoes rapid solidification; whereas the powder in the interior of the part is left unprocessed and undergoes densification during HIP post-processing. Figure 8 shows the interface between the can or skin and the core. The skin region exhibits a lamellar-type microstructure consistent with cast Ti-6Al-4V13 while the core exhibits a microstructure resulting from the coalescence of prior powder particles.
Table I. A Comparison of Hardness Values for Differently Processed Materials |
Material |
Processing |
Hardness |
Inconel 625 |
Solutionized and annealed |
50 HRA |
Alloy 625 |
Selective laser sintering and SLS/HIP |
52 HRA |
Ti-6Al-4V |
SLS-processed skin |
36 HRC |
Ti-6Al-4V |
Cast (grade C-5)14 |
39 HRC |
Ti-6Al-4V |
SLS/HIP-processed core |
35-37 HRC |
Ti-6Al-4V |
Glass-encapsulated HIP |
34-36.5 HRC |
|
Table II. The Tensile Properties of Ti-6Al-4V |
Processing |
Tensile Strength (MPa) |
Elongation (%) |
SLS/HIP |
1,116.9 |
5 |
Sidewinder Specifications |
765.3 |
5 |
Annealed15 |
896.3 |
10 |
Solution Treated16 |
1,034.2 |
8 |
Cast (grade C-5) |
896.3 |
6 |
|
Table III. Oxygen and Nitrogen Levels in Ti-6Al-4V |
Specimen |
Oxygen (wt.%) |
Nitrogen (wt.%) |
Sidewinder Specification |
0.25 max. |
|
PREPTM Ti-6Al-4V |
0.19 |
0.01 |
Argon-Atomized Ti-6Al-4V |
0.196 |
0.02 |
SLS/HIP 040898 Laboratory A Laboratory B |
0.250 0.255 |
0.019 0.037 |
SLS/HIP 042998 Laboratory A Laboratory B |
0.2485 0.200 |
0.073 0.016 |
SLS/HIP 050198 Laboratory A Laboratory B |
0.251 0.167 |
0.038 0.040 |
Rockwell hardness was evaluated for both materials and compared against conventionally processed material (Table I). For alloy 625, SLS processing to 98.5 percent density as well as HIP post-processing to 99.5 percent density both produced hardness values comparable to data for solutionized and annealed material.12 For Ti-6Al-4V, SLS processing of the skin to full density resulted in material having hardness values comparable to cast material (grade C-5). Hardness values for the SLS/HIP processed core agreed well with values for glass encapsulation HIP Ti-6Al-4V and did not differ much from the hardness values for the SLS-processed skin region.
Tensile specimens that conformed to ASTM E8 (small-size specimens 6.35 mm nominal diameter) were machined from the integrally canned Ti-6Al-4V cylinders fabricated by SLS and post-processed to full density by HIP (Table II). The results indicated that the tensile strength specification for the Sidewinder housing demonstration component was exceeded while minimum elongation was barely met.
Oxygen levels in commercially available titanium feedstock and finished product are critical for performance considerations. The oxygen levels can vary from 0.08-0.40 wt.%, depending on the particular application. The strength typically increases with increasing oxygen content at the expense of ductility. Lower oxygen levels improve ductility, fracture toughness, stress-corrosion resistance, and crack-growth resistance.17
Oxygen and nitrogen levels in SLS/HIP-processed Ti-6Al-4V specimens were determined by the ASTM E 1409-97 inert-gas fusion technique.18 The levels were compared against levels in the starting powder as well as against specifications for the Sidewinder housing component. The results of analyses conducted by two analytical labs are shown in Table III. All three SLS/HIP specimens for chemical analysis were taken from the core region of each part. The results indicate that the oxygen specification of the Sidewinder housing was barely met. However, it should be noted that both starting powders (PREPTM and argon-atomized) have 0.19 wt.% oxygen, and the powder used to fabricate each of the specimens had been recycled at least six times. This indicates that 100 ppm oxygen pickup, on the average, occurred during each SLS run. This is a notable result since it corresponds to the typical oxygen pickup observed in conventional metal can Ti-6Al-4V.19 To lower oxygen levels in SLS/HIP-processed Ti-6Al-4V and increase powder recyclability, extra-low interstitials grade Ti-6Al-4V powder may have to be used.
To validate the developed SLS/HIP process for Ti-6Al-4V, a scaled version of the guidance section housing for the AIM-9 Sidewinder missile was processed by SLS. This demonstration component, shown in Figure 9, was built at a 90 percent scale. The typical length scale on the component shown is 7.6 cm. Although this component was selected for SLS/HIP technology demonstration, metallographic evaluation of the as-SLS-processed component revealed full density (Figure 10). This microstructure is consistent with cast Ti-6Al-4V.
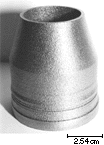 |
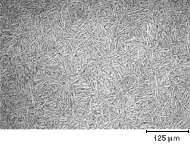 |
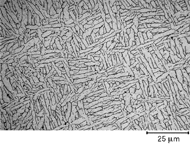 |
Figure 9. An SLS-processed Ti-6Al-4V demonstration component. |
Figure 10. The etched microstructure of a fully dense, SLS-processed Ti-6Al-4V demonstration component (Kroll's reagent). |
A preliminary evaluation of microstructure and mechanical properties (hardness and tensile) reveals that material processed by the SLS/HIP technique is equivalent to conventionally processed material. A simplified version of a Ti-6Al-4V demonstration component was successfully fabricated. Mechanical properties and oxygen levels in SLS/HIP-processed Ti-6Al-4V meet specifications for the demonstration component. Future work will focus on technology demonstration for alloy 625 and the further characterization of structure/property relationships, as well as the optimization of processing parameters for building speed, skin thickness, oxygen content, and HIP cycle time.
The authors acknowledge research funding provided by the U.S. Defense Advanced Research Projects Agency and Office of Naval Research under contract N00014-95-C-0139 titled "Low Cost Metal Processing Using SLS/HIP." Appreciation is given to Ronald C. Knight, contract program manager for Lockheed Martin Vought Systems, the prime contractor for this program.
1. S. Das et al., "Direct SLS Processing of Cermet Composite Turbine Sealing Components," Materials and Manufacturing Processes, 13 (3) (1998).
2. M.L. Griffith et al., "Freeform Fabrication of Metallic Components Using Laser Engineered Net Shaping (LENSTM)," Proc. Solid Freeform Fabrication Symposium 1996 (Austin, TX: University of Texas, 1996).
3. J. Mazumder et al., "The Direct Metal Deposition of H13 Tool Steel for 3-D Components," JOM, 49 (5) (1997), pp. 55-60.
4. G.K. Lewis et al., "Properties of Near-Net Shape Metallic Components Produced by the Directed Light Fabrication Process" Proc. Solid Freeform Fabrication Symposium 1997 (Austin, TX: University of Texas, 1997).
5. J.R. Fessler et al., "Laser Deposition of Metals for Shape Deposition Manufacturing," Proc. Solid Freeform Fabrication Symposium 1996 (Austin, TX: University of Texas, 1996).
6. F.G. Arcella et al., "Materials Characterization of Laser-Cast Titanium," Advances in Powder Metallurgy and Particulate Materials, part 15, 2 (1996) pp. 151-165.
7. S. Das et al., "Direct Selective Laser Sintering of High Performance Metals for Containerless HIP," Advances in Powder Metallurgy and Particulate Materials, part 21, 3 (1997) pp. 67-68.
8. R. Knight et al., "Metal Processing Using Selective Laser Sintering and Hot Isostatic Pressing" Proc. Solid Freeform Fabrication Symposium 1994 (Austin, TX: University of Texas, 1994).
9. G. Zong et al., "Direct Selective Laser Sintering of High-Temperature Materials," Proc. Solid Freeform Fabrication Symposium 1994 (Austin, TX: University of Texas, 1992).
10. D.W. Freitag et al., "Laser Directed Fabrication of Full Density Metal Articles Using Hot Isostatic Pressing," U.S. patent 5,640,667 (1997).
11. R.M. German, Powder Metallurgy Science (Princeton, NJ: MPIF, 1994), p. 57
12. INCONEL Alloy 625, Inco Alloys International (1985).
13. Metals Handbook, ninth ed., vol. 9 (Metals Park, OH: ASM, 1985), p. 466.
14. ASTM B-367-93, "Standard Specification for Titanium and Titanium Castings" (Philadelphia, PA: ASTM, 1993).
15. ASTM B 348-95a, "Standard Specification for Titanium and Titanium Alloy Bars and Billets" (Philadelphia, PA: ASTM, 1995).
16. Metals Handbook, ninth ed., vol. 3 (Metals Park, OH: ASM, 1993), p. 388.
17. R. Boyer et al., "Ti-6Al-4V," Materials Properties Handbook: Titanium Alloys (Materials Park, OH: ASM, 1994), p. 483.
18. ASTM E 1409-97, "Standard Test Method for Determination of Oxygen in Titanium and Titanium Alloys by the Inert Gas Fusion Technique" (Philadelphia, PA: ASTM, 1997).
19. C.F. Yolton, Crucible Research, personal communication (1998).
ABOUT THE AUTHORS
Suman Das earned his M.S. in mechanical engineering at the University of Texas at Austin in 1993. He is currently a Ph.D. candidate at the same school. Mr. Das is a member of TMS.
Martin Wohlert earned his M.S. in materials science and engineering at the University of Texas at Austin in 1997. He is currently a Ph.D. candidate at the same school. Mr. Wohlert is also a member of TMS.
Joseph J. Beaman earned his Sc.D. in mechanical engineering at the Massachusetts Institute of Technology in 1979. He is currently Anderson professor of manufacturing systems engineering at the University of Texas at Austin.
David L. Bourell earned his Ph.D. in materials science and engineering at Stanford University in 1979. He is currently Temple Foundation professor at the University of Texas at Austin. Dr. Bourell is also a member of TMS.
For more information, contact S. Das, University of Texas, Laboratory for Freeform Fabrication, ETC 5-160 C2200, Austin, Texas 78712-1063; (512) 471-5838; fax (512) 471-8727; e-mail das@sffoffice.me.utexas.edu.
Copyright held by The Minerals, Metals & Materials Society, 1998
Direct questions about this or any other JOM page to jom@tms.org.