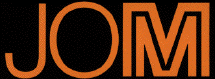 |
The following article is a component of the July 1999 (vol. 51, no. 7) JOM and is presented as JOM-e. Such articles appear exclusively on the web and do not have print equivalents.
|
Solidification: Overview
Investigating Solidification with the Laser-Engineered Net Shaping (LENSTM) Process
William Hofmeister, Melissa Wert, John Smugeresky, Joel A. Philliber, Michelle Griffith, and Mark Ensz
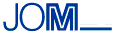 |
---|
TABLE OF CONTENTS |
---|
|
The laser-engineered net shaping (LENSTM) process is a laser-assisted, direct metal manufacturing process under development at Sandia National Laboratories. The process incorporates features from stereolithography and laser surfacing, using computer-aided design file cross sections to control the forming process. Powder-metal particles (less than 150 µm) are delivered in a gas stream into the focus of a Nd:yttrium-aluminum-garnet laser to form a molten pool. The part is then driven on an x/y stage to generate a three-dimensional part by layer-wise, additive processing. In an effort to understand the thermal behavior of the LENS process, in-situ high-speed thermal imaging has been coupled with microstructural analysis and finite-element modeling. Cooling of the melt is accomplished primarily by conduction of heat through the part and substrate, and depending on the substrate temperature and laser-input energy, cooling rates can be varied from 102 K s1 to 103 K s1. This flexibility allows control of the microstructure and properties in the part. The experiments reported herein were conducted on 316 stainless steel, using two different particle-size distributions with two different average particle sizes. Thermal images of the molten pool were analyzed to determine temperature gradients and cooling rates in the vicinity of the molten pool, and this information was correlated to the microstructure and properties of the part. Some preliminary finite-element modeling of the LENS process is also presented.
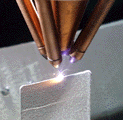 |
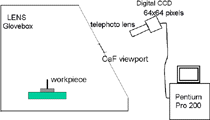 |
Figure 1. The LENS process performing a single-line build. |
Figure 2. A schematic of the thermal-imaging experimental setup. The thermal-imaging camera views the sample through a CaF viewpoint in the front of the LENS glovebox. |
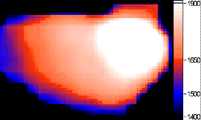 |
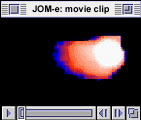 |
a |
b |
Figure 3. (a) A side view of a LENS line build. The image has been colorized to show temperature in Kelvin. (b) A video clip of the line build. (approx. 252K) |
Rapid prototyping using the stereo lithography process and plastic materials has enjoyed great success. The natural extension of this process to structural materials has been accomplished with the selective laser sintering (SLS) process. In addition, various methods of laser cladding and laser-assisted surface modification are also being practiced.
Laser-engineered net shaping (LENSTM) builds on the SLS process with a few notable exceptions. Instead of bonding material in a bed of powder, the powder is delivered in a gas jet through nozzles. Solid material is formed by solidification of a molten pool, thus forming a fully dense, free-standing deposit. LENS development is the subject of a cooperative research and development agreement (CRADA) at Sandia National Laboratory. The technology is being commercialized by several of the CRADA participants, including Optomec Design Company, MTS Systems, and AeroMet Corporation.
A photograph of a single-pass line built of 316 stainless steel is shown in Figure 1. The LENS powder-delivery system consists of a commercial powder feeder that delivers powder into a gas-delivery system by way of the four nozzles shown in the figure. The Nd:YAG laser beam is delivered along the z-axis in the center of the nozzle array and focused by a lens in close proximity to the work piece. The work piece is driven in the x and y directions by a motorized stage. Moving the lens and powder nozzles in the z direction controls the height of the laser and powder focus.
In order to understand the development of structure and properties in the solidified material, it is important to know the thermal gradients and cooling rates in and around the molten pool. These gradients control the morphology and scale of the first solid to form and are a primary factor in determining the properties of the sample. Since the heat from the melt pool is conducted primarily through the substrate, this thermal information can be used as a boundary condition in heat-transfer models to determine the complete thermal history of the sample.
Thermal measurements, microstructural studies, and modeling can be combined to develop process parameters to control microstructural development and tailor the properties of samples for particular applications. As a first step, thermal imaging was employed in monitoring line builds of 316 stainless steel at a variety of laser powers for -100 mesh powder and +100325 mesh powder. The microstructures of the deposits were investigated and correlated to the thermal conditions at solidification. Finally, a finite-element model of the line-build process was developed to determine the thermal history of the sample in areas that are not accessible to thermal-imaging measurements.
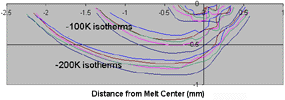 |
Figure 4. Isotherms at 100 K and 200 K below the melt on the side wall of a line build. Each color corresponds to a different image frame of data. The coordinates of the laser spot are (0,0) and the sample is moving from right to left. |
High-speed thermal imaging has been used in the study of solidification kinetics and droplet impact and spreading. For the LENS studies, a digital 64 x 64 pixel CCD video camera was employed. This camera digitizes monochrome images to 12 bits and passes the frame data to a personal computer for storage and processing. A telephoto lens and a broad band-pass filter centered at 650 nm were used in the image path. The camera, lens, and filter were calibrated for temperature measurement with a tungsten-strip lamp radiance source obtained from the National Institute of Standards and Technology. The experimental setup is shown in Figure 2. The powder and laser focus do not move in the x-y plane so that the build could be tracked by adjusting the tilt of the camera. The camera angle was measured with a clinometer and was adjusted as the build progressed. Images were taken at up to 990 frames per second. At the end of each set of imaging experiments, a molten pool was formed with no translation of the work piece to observe the freezing plateau for in-situ calibration.
A typical side view image of a line build is shown in Figure 3a. The image has been converted to temperature and colorized according to the adjacent scale. The angle of the image to the x-y plane is approximately 30° so that the top surface as well as the front face of the build is visible. Since the angle of the plane of the camera to the sample is known, the distances and temperatures can be corrected for the angle of view. Clicking on Figure 3b will play a movie of the build.
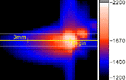 |
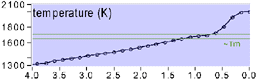 |
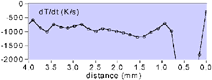 |
a |
b |
c |
Figure 5. (a) A thermal image of the line build with corresponding graphs of (b) the temperature distribution along the yellow cursor and (c) the cooling rate. |
Images of the kind shown in Figure 3 were analyzed to determine the isotherms on the side wall of the build. The information from several frames is presented in Figure 4, with each frame represented by a different color. The melt isotherm has a length of 0.75 mm.
The gradients in the previous build layer below the laser are as high as 400 K mm1 and taper off to less than 200 K mm1 in the trail of the molten pool. Since the sample is moving in the x-direction, it is possible to scale these gradients with the sample velocity to derive cooling rates for material deposited in the x-direction. For example, in Figure 5a the image is shown at the top, and the temperature along the yellow cursor line from the begin mark and to the left of that mark is shown in Figure 5b. This temperature graph shows the temperature distribution along the direction of travel. This sample was built at a traverse velocity of 7.62 mm s1, so that the resulting cooling rate can be calculated (dT/dx*dx/dt) and is displayed in Figure 5c. The highest cooling rates are in the liquid ahead of the solid-liquid interface. At the melting temperature and below, the cooling rates approach 103 K s1.
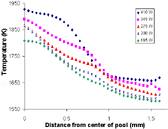 |
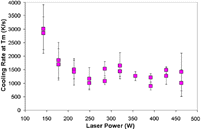 |
Figure 6. Thermal profiles from the center of the molten pool along the direction of translation for several different laser power settings. |
Figure 7. Cooling rates calculated from a large series of images as a function of laser power. |
The thermal profiles of the build reveal interesting nonlinearities in the build process. Profiles similar to those in Figure 5 were compiled for line builds as a function of laser power. These are presented in Figure 6 for the -100/+325 mesh powder. The laser power for each profile is shown in the legend. The molten pool size increases with power up to 275 W. Above 275 W the energy of the laser drives the pool temperature up without a significant change in the length of the molten zone. Note also that the slopes of the temperature profiles outside the molten zone generally increase as the power decreases. Higher power results in more bulk heating of the sample away from the molten zone. This results in a lower cooling rate in the solid sample after solidification. For example, the cooling rate of the 275 W sample 0.5 mm from the solid-liquid interface is twice that of the 410 W sample. The initial scale of the microstructure, however, should be determined by the cooling rate at the solid-liquid interface.
A complete series of line builds was analyzed to determine the cooling rate at the solid-liquid interface. These determinations are shown in Figure 7. At the interface, the cooling rates are substantially higher at the low power levels and remain fairly constant at the higher powers. Thus, the highest quench rates are available at the lowest power, when the molten zone is small. As the laser power is increased, the quench rate at the interface settles at 1,0001,500 K s1. In the solid, however, the cooling rate at the highest powers is lower so that one may expect a more coarsened microstructure due to grain growth.
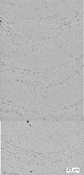 |
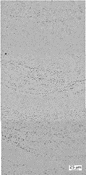 |
 |
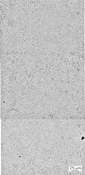 |
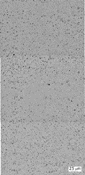 |
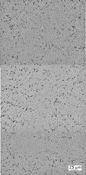 |
a |
b |
c |
d |
e |
f |
Figure 8. Solidification microstructures of LENS-processed 316 stainless steel at (a) 115 W, (b) 165 W, (c) 200 W, (d) 275 W, (e) 345 W, and (f) 410 W. |
The solidification microstructures of LENS-processed 316 stainless steel are complex and varied as one might expect in a rapid solidification structure. In general, columnar structures predominate near the interface of the molten pool. This feature is clearly seen in the series of microstructures presented in Figure 8. The remaining regions of the samples have a cellular structure. The micrographs are montages of optical micrographs taken from a cross section of the samples processed with the 100/+325 mesh powder. The z direction or height of the build is vertical in the micrographs, and the width of the micrographs is the y direction. An oxalic-acid electrolytic etch was used to delineate the austenite cell boundaries.
Table I. The Mean Intercept Length Between Cell Boundaries for +100/325 Mesh Powder |
---|
Laser Power (W) |
Mean Intercept Length (µm) |
410 |
8.68 |
345 |
8.55 |
275 |
7.12 |
200 |
6.46 |
165 |
4.63 |
115 |
3.25 |
The dendrites and cells at the lower power levels are finer. At the higher powers, significant dendrite coring and cell substructure can be seen. A comparison of the microstructural scale of these samples was obtained by measuring the mean intercept length of cell boundaries with a Hillard circle. These results are presented in Table I. The uncertainty of these determinations is about ten percent.
The macrothermal characteristics during part fabrication are important particularly as they apply to solid-state transformations, dimensional accuracy, and residual stress in built components. Initial work has focused on finite-element analysis (FEA) methods with element birthing to simulate LENS processing. Thermal behavior during the fabrication of a thin wall has been modeled. The material properties are based on generic stainless steel, and for the initial studies, only conduction is used for thermal transfer. The substrate (Figure 9) is held at 300°C throughout the simulation, whereas thermocouple data indicate that the substrate reaches this steady state when the build is approximately 6.3 mm tall. The wall was made of 1.27 mm3 elements for a 25.4 mm long, 76.2 mm tall wall.
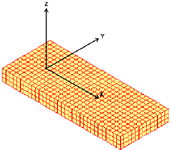 |
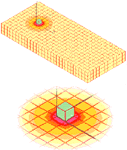 |
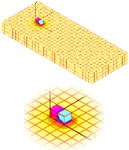 |
|
a |
b |
Figure 9. An FEA model of the substrate. |
Figure 10. The thermal distribution (a) after the placement of the first element on the substrate and (b) after the addition of the second element. |
The first element (Figure 10a) was positioned onto the substrate with a set initial temperature at all of the eight nodes. These nodes were set at the melting temperature (1,377°C) for one set of FEA experiments and at a superheat temperature (1,627°C) for another set following the results of the imaging experiments. The element was held at the set temperature for the amount of time the laser dwells at this position, and then the model computed the final temperature values or nodes in the model (element plus substrate) and stored the data.
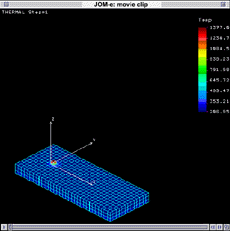 |
Figure 11. A video clip simulation of a wall build. (approx. 2.5MB) |
For subsequent elements (Figure 10b), the model used the results from the previous step as the initial condition for each new element birth. Once again, the new element was set at the initial temperature, the time step was calculated, and the temperature data for all elements were stored. This was repeated for all birthing events until the geometry was complete. The entire simulation of the wall build has been assembled in a video (Figure 11). As seen in the movie, large thermal gradients exist in the LENS process. Near the molten pool, the energy is concentrated, and the thermal gradients are steep. Far from the molten pool, the gradients reach a steady-state thermal front, where the base is the main conduction path for the energy. A clear picture of LENS thermal behavior can be obtained by understanding these gradients and refining the model with experimental verification.
Meshing algorithms are being developed to decrease the processing time per element birth sequence in order to utilize these techniques for complex components. FEA will be used to determine where the thermal gradients exist, especially with respect to complex part geometry to reduce distortion and thermal stresses in part fabrication. Furthermore, understanding the thermal behavior will clarify the microstructural effects of these gradients on final properties for LENS-fabricated components.
Sandia is a multiprogram laboratory operated by Sandia Corporation, a Lockheed Martin Company, for the U.S. Department of Energy under contract number DE-AC04-94AL85000.
William Hofmeister is with the Department of Chemical Engineering, Vanderbilt University. Melissa Wert is with the materials science and engineering program at Vanderbilt University. John Smugeresky, Joel A. Philliber, Michelle Griffith, and Mark Ensz are with Sandia National Laboratories.
For more information, contact W.H. Hofmeister, Vanderbilt University, Department of Chemical Engineering, 24th and Garland Avenue, Nashville, Tennessee 37212; (615) 322-7053; (615) 343-0466; e-mail ho@vuse.vanderbilt.edu.
Copyright held by The Minerals, Metals & Materials Society, 1999
Direct questions about this or any other JOM page to jom@tms.org.