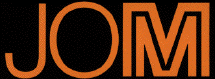 |
The following article is a component of the October 1997 (vol. 49, no. 10) JOM
and is presented as JOM-e. Such articles appear exclusively
on the web and do not have print equivalents.
|
Overview
The Challenge of Precursor Compounds in the MOCVD of Oxides
Ren Xu
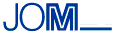 |
---|
CONTENTS |
---|
|
The technology of metalorganic chemical vapor deposition (MOCVD) for oxides has experienced explosive advances in the past ten years due to popular interests in oxide superconducting, ferroelectric, and dielectric materials. The characteristics of deposited oxide films show crucial dependence on the selection of precursor compounds, deposition temperature and environment, and kinetic factors such as the precursor partial pressure and flow rate. A simple classification of precursor compounds and discussion on their physical and chemical characteristics within the context of vapor deposition can be useful for MOCVD practitioners.
The technology of metalorganic chemical vapor deposition (MOCVD) for oxides has demonstrated explosive advances in the past ten years because of popular interests in oxide superconducting, ferroelectric, and dielectric materials. These oxide compounds, with the exception of simple dielectrics such as SiO2 and Ta2O5, tend to be complex in composition and structure. They often involve metallic elements with wide-ranging size, electronegativity, and oxidation states that necessarily require complex organic ligands to form volatile, yet thermally stable, precursor compounds. Consequently, the method of precursor delivery, as well as the type of deposition reactions, vary drastically between components for any multicomponent oxide. The characteristics of deposited oxide films thus show crucial dependence on the selection of precursor compounds, deposition temperature, deposition environment, and kinetic factors such as the precursor partial pressure and flow rate. While the variety of precursor compounds known today is large and the processes of film deposition are complex, a simple classification of precursor compounds and discussion on their physical and chemical characteristics within the context of vapor deposition can be useful to MOCVD practitioners.
Metalorganic compounds suitable as precursors for most main-group and transition-metal elements are now available from a number of commercial sources. When not available or when stringent requirements are imposed, precursors are readily prepared in laboratories for immediate use in vapor depositions. Table I is a partial list of some examples of commercially available or laboratory-prepared precursor compounds used in the MOCVD of oxides.1-28 There are four categories of metalorganic compounds frequently used: b-diketonates, such as 2,2,6,6-tetramethyl-3,5-heptanedionate (thd) and 2,4-pentanedionate (also known as acetoacetonate, acac); alkoxides, such as ethoxide (OEth), isopropoxide (i-OPr), and butoxide (n-OBut); alkylmetal, such as ethylzinc and phenylbismuth; and carboxylates, such as benzoate and ethylhexanoate (eha). The metallic elements are usually in their correct oxidation state for
-diketonates, alkoxides, and carboxylates; thus, they do not require the presence of oxygen during deposition.
Table I. Metalorganic Compounds for Oxide Deposition |
Metal Equipment |
Diketonate |
Alkoxide |
Alkylate/Carboxylate |
Oxide Examples |
Main Group |
IA (Li) |
thd1,2 |
-OEth3,4,n-OBut5 |
LiTaO3,LiNbO3 |
|
IIA (Mg,Sr,Ba) |
thd6-9 |
-OEth10 |
|
Bi2Sr2Ba2Cu3Ox YBa2Cu3O6+ ,MgAl2O4 |
IIIA (Al,In) |
acac11 |
-OEth10,i-OPro12 |
Benzoate13 |
In2O3,Al2O3,MgAl2O4 |
IVA (Si,Sn,Pb) |
thd14 |
-OEth15 |
TEPb16,eha17 |
SiO2,SnO2,PbTiO3,Pb(Zr,Ti)O3 |
VA (Bi) |
|
|
Phenyl9 |
Bi2Sr2Ba2Cu3Ox |
Transition Metal |
IIIB (Y,La,Eu) |
thd6-8,18,19,tfa20 |
|
|
YBa2Cu3O6+ . PLZT, Eu2O3 |
IVB (Ti,Zr) |
thd21,tfa22acac23 |
n-OBut20 |
|
|
VB (Nb,Ta) |
|
n-OBut5,-OEth2-4 |
LiTaO3,LiNbO3,(Ta,Nb)2O5 |
|
VIB (Cr) |
acac24 |
|
|
Cr2O3 |
VIIB (Mn) |
acac25 |
|
|
Mn2O3,Lax(Sr,Ca)yMnO3 |
VIIIB (Co) |
acac26 |
|
|
LaSrCoO3 |
IB (Cu) |
thd6-9 |
|
|
Bi2Sr2Ba2Cu3Ox |
IIB (Zn) |
acac27 |
|
Ethyl28 |
ZnO |
When alkylmetals are used in MOCVD, an oxidizing environment must be used. This is sometimes dangerous because of the ignitability and toxicity of most alkylmetal compounds. The use of water vapor in the MOCVD of oxide has a relatively long history dating back to the early 1970s. The catalytic effect of H2O in alkoxide pyrolysis significantly enhances the deposition rate as well as the deposition quality of oxides. Al2O3, Nb2O5, Sb2O3, TiO2, and ZrO2 films were deposited by Sladek et al.12 through vapor-phase hydrolysis of alkoxides. Matievic et al. used an atmospheric apparatus to produce aerosol particles of TiO229 and Al2O3.30 Recently, the effect of H2O vapor in enhanced quality at lower deposition temperatures for ZrO2,23 YSZ,20 and LiTaO35 was noted. Laser-assisted pyrolytic deposition was used to induce oriented crystallization by surface nucleations.8
Due to the low volatility inherent to common precursors, most oxide depositions are achieved at reduced pressures, ranging from a few torr to a few ten torrs. Occasionally, atmospheric vapor deposition becomes feasible when the precursor is sufficiently volatile. Such is the case for ZnO using Zn(acac)2 as a precursor.27 The vapor pressure of precursor compounds at various temperatures has far-reaching consequences beyond the operating pressure range for the depositions. One important aspect of multicomponent oxide deposition is the control of vapor-phase compositions. Since the majority of the oxide systems of present interest have melting points much higher than the typical deposition temperature, insufficient diffusion results in nonequilibrium depositions. Consequently, the composition of the deposited films is highly dependent on the individual precursor partial pressure immediately above the substrate surface. Presently, little effort has been devoted to in-situ monitoring and control of the vapor-phase composition in MOCVD experiments. The common practice is to use the composition-analysis result on deposited films as a qualitative guide for the adjustment of relative carrier gas-flow rate and precursor evaporation temperature in order to reach acceptable deposition conditions. The lack of a viable analytical technique with sufficient accuracy has hindered the improvement of film qualities for practical device applications. On the other hand, there have been numerous reports on the sensitivity of film optical, electrooptical, and electrical properties on compositional,31,32 structural,33 and morphological34 defects in oxides.
Metal alkoxides are readily prepared by reactions of metal or metal chlorides with appropriate alcohols. For conversion from lower alkoxides to higher alkoxides, alcoholysis reactions are carried out through simple dry-atmosphere reflux and distillations.35 For example, alkali metal reacts directly with anhydrous alcohol in inert and dry atmosphere to produce hydrogen gas and a transparent alkoxide solution in parent alcohol:
Li(Na,K) + HOC2H5 Li(Na,K)OC2H5 + H2 | (1) |
Subsequent alcoholysis reactions convert the lower alkoxides to higher alkoxides.
LiOC2H5 + HOC4H9 LiOC4H9 + HOC2H5 | (2) |
When subjected to distillation, the lower alcohol evaporates first, leaving behind the higher alkoxide solution in higher alcohol. Similar conversions can be performed to other metal alkoxides when a commercial source is not identified for the particular precursor.
b-diketonates are readily prepared by directly refluxing the respective b-diketones with lower alkoxides of the metal element in appropriate solvent. For example, Al(OC2H5)3 and Al(i-OC3H7)3 react with acetylacetone in benzene-yielded trisderivatives:35
Al(OR)3 + 3acacH Al(acac)3 + 3HOR | (3) |
Carboxylates are generally prepared by directly refluxing the carboxylic acid with lower alkoxides and subsequently distilling out the alcohols. Alkylmetals are generally available from commercial sources and are too dangerous to prepare in laboratories.
Liquid precursor delivery systems were developed, tested, and recently became commercially available for MOCVD applications.26 Such an apparatus is generally compatible with most MOCVD reactors and their operating conditions. Metalorganic precursors with low volatility and poor thermal stability can be handled collectively with some flexibility in the component concentrations in the solutions, if proper solvents are selected. Such a liquid-injection method is similar to spray pyrolysis in that the composition of the vapor-phase species can be prescribed to a certain degree. Further, the component concentration in the solution is preserved precisely until the liquid is quickly evaporated upon hitting a flash evaporator, which consists of a heated stainless-steel surface situated adjacent to the separately heated substrate.10 In other cases, the liquid is directly injected at repeated pulses at quantities equivalent to a single molecular layer deposition per pulse.4 These techniques were successfully employed in the deposition of MgAl2O4 and LiTaO3 from nonvolatile and thermally unstable alkoxide complexes MgAl2(OC2H5)810 and LiTa(OC2H5)6.4 The general applicability of such liquid-delivery evaporation systems was demonstrated for other oxide systems such as BaTiO3, YBa2Cu3O7-d, YSZ, LaSrCoO3, and copper using mixtures of respective precursors in the solution.26
It turns out that many heterometallic alkoxide complexes, called double alkoxides, are available at appropriate metal-to-metal ratios for a number of important multicomponent oxide systems. The thermal stability and volatility of these double alkoxides vary. In solutions using parent alcohol as a solvent, double alkoxides have sufficient stability to be recrystallized or to show sharp transitions in potentiometric titrations between single alkoxides.35 Hence, these double alkoxides in their solution form are ideal precursors for the deposition of the respective multicomponent oxides using liquid precursor delivery with flash evaporators. Table II shows a partial list of some examples of double alkoxides matched with the respective multicomponent ferroelectric oxides.
Table II. Examples of Double Alkoxides |
Double Alkoxide |
Vapor Pressure (°C/mm Hg)* |
Oxide |
Application |
LiTa(OEt)6 |
230/0.2 |
LiTaO3 |
Pyroelectricity, electrooptics, and second harmonic generation |
LiTa(i-OPr)6 |
160-180/0.1 |
LiTa(t-OBut)6 |
110-120/0.1 |
|
LiNb(i-OPr)6 |
<140/0.2 |
LiNbO3 |
Electrooptics and surface acoustic device |
LiNb(t-OBut)6 |
110-120/0.1 |
|
KNb(i-OPr)6 |
<200/0.8 |
KNbO3 |
Electrooptics and nonlinear optics |
KNb(t-OBut)6 |
<200/0.8 |
|
Ba[Nb(i-OPr)6]2 |
170-180/0.1 |
Ba4+xNa2-2xNb10O30 |
Nonlinear optics and second harmonic generation |
NaNb(t-OBut)6 |
110-120/0.1 |
|
LiNb(t-OBut)6 |
110-120/0.1 |
K3Li2Nb5O15 |
Nonlinear optics and Electrooptics |
KNb(t-OBut)6 |
<200/0.8 |
|
KNb(t-OBut)6 |
<200/0.8 |
K3Li2(TaxNb1-x)5O15 |
Electrooptics |
KTa(t-OBut)6 |
<200/0.8 |
LiNb(t-OBut)6 |
110-120/0.1 |
LiTa(i-Opr)6 |
160-180/0.1 |
|
KNb(t-OBut)6 |
<200/0.8 |
KTa1-xNbxO3 |
Second harmonic generation, electrooptics and hollographic storage |
KTa(t-OBut)6 |
<200/0.8 |
|
Sr[Nb(i-OPr)6]2 |
210-220/0.1 |
SrxBa1-xNb2O6 |
Pyroelectricity, electrooptics, and hollographic storage |
Ba[Nb(i-OPr)6]2 |
170-180/0.1 |
*Data according to Bradley et al.35 |
For complex precursors such as LiTa(OR)6 with limited stability, where R = CnH2n+1 (n = 1-6), the evaporation behavior can generally be considered as two competing processesthe simple evaporation of complex molecule and the decomposition-evaporation of the precursor. For the simple case of the LiTa(OR)6 system, the competing processes can be written as
LiTa(OR)6 (liquid) LiTa(OR)6(vapor) | (4) |
LiTa(OR)6(liquid) LiOR(solid) + Ta(OR)5(liquid) | (5) |
Ta(OR)5(liquid) Ta(OR)5(vapor) | (6) |
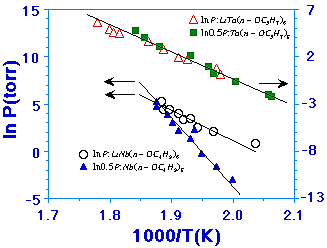 |
Figure 1. The vapor pressure-temperature behavior for the LiNb(n-OBut)6:Nb(n-OBut)5 system (scale on left side) and the LiTa(n-OPr)6:Ta(n-OPr)5 system (scale on right side).
|
The composition of the vapor-phase species is determined by the stability of the double alkoxide LiTa(OR)6 and the relative volatility between LiTa(OR)6 and Ta(OR)5. For unstable LiTa(OR)6, the liquid phase can be considered a simple mixture of LiOR and Ta(OR)5. Since LiOR is mostly ionic with a very low vapor pressure as compared to Ta(OR)5,35,36 the vapor-phase composition is simply determined by the relative volatility of LiTa(OR)6 to that of Ta(OR)5. A completely decomposed LiTa(OR)6 has Ta(OR)5 in a solution at 50 mol.% concentration. It is, thus, informative to compare the vapor pressure versus temperature behavior of LiTa(OR)6 with that of Ta(OR)5 in a 50 mol.% solution. Experimentally, this can be performed for all varieties of double alkoxides and the respective volatile single alkoxides. Figure 1 shows the results of such measurements37 for LiNb(n-OC4H9)6, Nb(n-OC4H9)5, LiTa(n-OC3H7)6, and Ta(n-OC3H7)5. For the LiNb(n-OC4H9)6 and Nb(n-OC4H9)5 systems, the vapor pressure-temperature behaviors are sufficiently different between the double and single alkoxide, indicating that the LiNb(n-OC4H9)6 is structurally different from a simple solution of Li(n-OC4H9) in Nb(n-OC4H9)5. On the other hand, the vapor-pressure behavior for LiTa(n-OC3H7)6 and Ta(n-OC3H7)5 are identical within the errors of the experiment, suggesting that the structure of LiTa(n-OC3H7)6 is probably the same as a 50 percent Raultian solution of Ta(n-OC3H7)5.
The vapor-pressure behaviors for five double alkoxide-single alkoxide pairs was studied. In all cases, an Arrhenius relationship with temperature was found; the Arrhenius coefficients for each compound can be found by linear regression. It should be noted that the relative volatility between double and single alkoxides can be used as an indicator of composition of the vapor-phase species. Consider a partially stable double alkoxide LiTa(OR)6 with 50 percent decomposition at the evaporation temperature. The vapor-pressure ratio between Ta(OR)5 and LiTa(OR)6 can be calculated on the basis of the Arrhenius equation
Using the constants found from the linear fit of vapor pressure-temperature data, the fraction excess Ta(OR)5 in the vapor phase with respect to LiTa(OR)6 can be estimated as
K =  | (8) |
InK = InP = InPTa(OR)5 - InPLiTa(OR)6 | (9) |
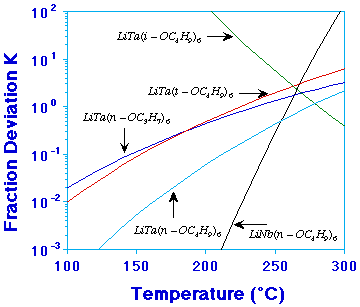 |
Figure 2. The nonstoichiometry factor in the temperature range 100-300°C for five double-alkoxide systems.
|
Figure 2 shows the calculated nonstoichiometry factor K (The fraction deviation from stoichiometric composition proposed earlier) for five alkoxide systems studied. It is apparent that LiNb(n-OC4H9)6 and LiTa(n-OC4H9)6 are more likely to evaporate stoichiometrically.
The stability of double-alkoxide complexes is apparently maintained through a different mechanism than for single alkoxides. For single alkoxides, a branched alkoxyl group generally favors thermal stability. Better charge screening is expected out of branched alkoxyl groups for alkaline and alkaline-earth elements with strong ionic characters. Double alkoxides, on the other hand, appear to evaporate in the form of dimers, as suggested by mass spectrometric study of double-alkoxide vapor.36 A linear, long-chain alkoxyl group favors the formation of dimers with the long hydrocarbon chain wrapped around to provide charge screening to the alkaline elements. Such an argument appears to be in agreement with our observations in the LiNb(n-OC4H9)6 and LiTa(n-OC4H9)6 systems.
The composition of sublimate for the LiTa(n-OC4H9)6 system was analyzed by lattice-parameter measurement. Figure 3 shows a series of standard samples prepared in this study. Lattice parameters were measured on annealed samples with varying compositions. Silicon powder was mixed prior to x-ray diffraction as an internal standard. The calibration curve was obtained by scaling the Li:Ta ratio 1:1 sample using JCPDS card number 29-836. A similar standard prepared by Barns et al.38 is also shown as reference. The individual lattice parameter of a stoichiometric sample by Abrahams et al.39 is indicated in the figure by an arrow, and the lattice parameter of a Puratronic® standard sample from Johnson Matthey Company is also shown. The calcined sublimate from LiTa(1-C4H9)6 showed a lattice parameter of 5.1518, corresponding to Li1.01±0.02Ta0.99±0.02O2.98±0.06. The evaporation of LiTa(n-OBut)6 is, thus, stoichiometric within the composition detection limit by the x-ray lattice-parameter method. Stoichiometric and epitaxial LiTaO3 films were deposited from direct evaporation of the LiTa(n-OBut)6 precursor, and such a deposition process was referred to as the autostoichiometric vapor deposition.37
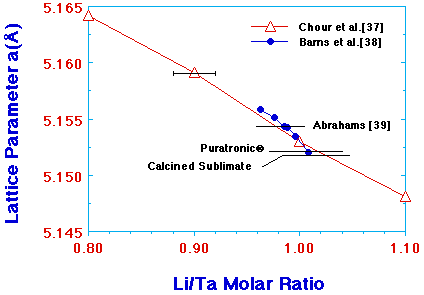 |
Figure 3. The stoichiometric evaporation of LiTa(n-OBut)6 as evidenced by lattice-parameter measurement.
|
It should be recognized that, between the preferred characteristics of high volatility and high thermal stability, difficulty remains in identifying appropriate metalorganic compounds as precursors. Liquid injection and flash evaporators are important advances because a wider variety of metalorganic compounds can now be used in the MOCVD of oxides. However, this technique is limited to the precursors that have sufficient solubility in some solvents. In addition, when liquid is directly injected on a substrate surface, the uniformity and the density of the layer, and ultimately the compositional and structural perfection of the deposited layer, become suspect. There have not been attempts in active monitoring and control of vapor-phase precursor compositions.
From a chemistry standpoint, the ultimate role of a precursor compound should be the capability to deliver metallic elements to the deposition site at a precisely controlled, constant dosage. The precision in dosage control should reach the parts-per-million to parts-per-billion level, if not that of a single molecular level. The precursor should play an active role in regulating the relative ratio between metal elements, and the single-precursor approach is an important step in that direction.
Finally, the precursor compound should permit at least one reaction mechanism through which a clean deposition reaction with little contamination can be carried out. The MOCVD of oxides has advanced to the present stage where precursor compounds suitable for deposition can be identified, purchased, or prepared on demand; when it comes to the quality of films deposited, much remains to be explored.
The experiments were performed by my students R.C. Zhang and K.W. Chour. Funding for the autostoichiometric vapor deposition of LiTaO3 was provided by the U.S. National Science Foundation, grant number DMR95-26165. Partial support was also provided by a University of Utah faculty research grant.
1. B.J. Curtis and H.R. Brunner, "The Growth of Thin Films of Lithium Niobate by Chemical Vapor Deposition," Mat. Res. Bull., 10 (1975), pp. 515-520.
2. A.A. Wernberg et al., "Epitaxial Growth of Lithium Niobate Thin Films from a Single Source Organometallic Precursor Using Metalorganic Chemical Vapor Deposition," Appl. Phys. Lett., 62 (9) (1993), pp. 946-948.
3. A.A. Wernberg, G.H. Braunstein, and H.J. Gysling, "Improved Solid Phase Epitaxial Growth of Lithium Tantalate Thin Films on Sapphire, Using a Two-step Metaloganic Chemical Vapor Deposition Process," Appl. Phys. Lett., 63 (19) (1993), pp. 2649-2651.
4. H. Xie and R. Raj, "Epitaxial LiTaO3 Thin Film by Pulsed Metalorganic Chemical Vapor Deposition from a Single Precursor," Appl. Phys. Lett., 63 (23) (1993), pp. 3146-3148.
5. K.W. Chour and R. Xu, "Autostoichiometric Vapor Deposition: Part II. Experiment," J. Mater. Res., 10 (10) (1995), pp. 2542-2547.
6. M. Schieber et al., "Comparison of Thin Films of YBa2Cu3O7-d Deposited by Physical (Laser Ablation) and Chemical (OMCVD) Methods for Device Applications," J. Cryst. Growth, 115 (1991), pp. 31-42.
7. H. Hayashi et al., "Preparation of YBa2Cu4O8 Thin Films by MOCVD," J. Cryst. Growth, 115 (1991), pp. 782-787.
8. T. Ushida et al., "Effect of UV Light Irradiation on the A-Axis Orientation Mechanism for YBa2Cu3O7-d Films Prepared by Laser Metalorganic Chemical Vapor Deposition," Jpn. J. Appl. Phys., 31 (1992), pp. L608-L611.
9. H. Yamasaki et al., "Critical Current Density of High-Quality Bi2Sr2Ca2Cu3Ox Thin Films Prepared by Metalorganic Chemical Vapor Deposition," J. Appl. Phys., 72 (7) (1992), pp. 2951-2957.
10. J. Zhang et al., "Single Molecular Precursor Metalorganic Chemical Vapor Deposition of MgAl2O4 Thin Films," J. Mater. Res., 9 (6) (1994), pp. 1333-1336.
11. J. Nishino et al., "Conductive Indium-Doped Zinc Oxide Films Prepared by Atmospheric-Pressure Chemical Vapor Deposition," J. Am. Ceram. Soc., in press.
12. K.J. Sladek and W.W. Gilbert, "Low Temperature Metal Oxide Deposition by Alkoxide Hydrolysis," Proc. 3rd Intern. Conf. on Chem. Vap. Deposition (Publisher's City: Publisher, 1972) pp. 215-231.
13. A.F. Depp et al., "Synthesis and Decomposition of a Novel Carboxylate Precursor to Indium Oxide," Mat. Res. Soc. Symp. Proc., vol. 335 (Pittsburgh, PA: MRS, 1994), pp. 227-232.
14. C.H. Peng and S.B. Desu, "Low Temperature Metalorganic Chemical Vapor Deposition of Perovskite Pb(ZrxTi1-x)O3 Thin Films," Appl. Phys. Lett., 61 (1) (1992), pp. 16-18.
15. A.C. Adams and C.D. Capio, "The Deposition of Silicon Dioxide Films at Reduced Pressure," J. Electrochem. Soc., 126 (6) (1979), pp. 1042-1046.
16. B.S. Kwak, E.P. Boyd, and A. Erbil, "Metalorganic Chemical Vapor Deposition of PbTiO3 Thin Films," Appl. Phys. Lett., 53 (18) (1988), pp. 1702-1704.
17. J. Mantese et al., Mat. Res. Soc. Bull., 14 (10) (1989), p. 48.
18. G. West and K.W. Beeson, "Low-Pressure Metalorganic Chemical Vapor Deposition of Photoluminescent Eu-doped Y2O3 Films," J. Mat. Res., 5 (1990), pp. 1573-1580.
19. M. Shimizu and T. Shiosaki, "MOCVD of Ferroelectric Pb(Zr,Ti)O3 and (Pb,La)(Zr,Ti)O3 Thin Films for Memory Device Applications," Mat. Res. Symp. Proc., vol. 361 (Pittsburgh, PA: MRS, 1995), pp. 295-305.
20. K.W. Chour, J. Chen, and R. Xu, "Metalorganic Vapor Deposition of YSZ Electrolyte Layer for Solid Oxide Fuel Cell Applications," Thin Solid Films, in press.
21. J. Si, S. Desu and C.Y. Tsai, "Metalorganic Chemical Vapor Deposition of ZrO2 Films Using Zr(thd)4 as Precursor," J. Mater. Res., 9 (7) (1994), pp. 1721-1727.
22. C.S. Hwang and H.J. Kim, "Deposition and Characterization of ZrO2 Thin Films on Silicon Substrate by MOCVD," J. Mater. Res., 8 (6) (1993), pp. 1361-1367.
23. J.S. Kim, H.A. Marzouk, and P.J. Reucroft, "Deposition and Structural Characterization of ZrO2 Films by Chemical Vapor Deposition," Thin Solid Films, 254 (1995), pp. 33-38.
24. R.S. Broorse and J.M. Burlitch, "MOCVD Route to Stable, Oxygen-Rich, Chromium Oxide Films and Their Conversion to Epitaxial Cr2O3" Chem. Mat., 6 (1994), pp. 1509-1515.
25. D.B. Studebaker et al., "Metalorganic Chemical Vapor Deposition of Lax(Ca, Sr)yMnO3 Films," Mat. Res. Soc. Symp. Proc., vol. 475 (Pittsburgh, PA: MRS, in press).
26. R.A. Gardiner, P.C. Van Buskirk, and P.S. Kirlin, "Liquid Delivery of Low Vapor Pressure MOCVD Precursors," Mat. Res. Soc. Symp. Proc., vol. 335 (Pittsburgh, PA: MRS, 1994), pp. 221-226.
27. K. Kamata et al., "Rapid Formation of Zinc Oxide Films by an Atmospheric Presure Chemical Vapor Deposition Method," J. Am. Ceram. Soc., 77 (2) (1994), pp. 505-508.
28. D. Shimizu et al., "Photo-MOCVD of ZnO Epitaxial Films," J. Cryst. Growth, 99 (1990), pp. 399-402.
29. M. Visca and E. Matijetic, "Preparation of Uniform Colloidal Dispersions by Chemical Reactions in Aerosols," J. Colloid. Interface Sci., 68 (2) (1979), pp. 308-319.
30. B.J. Ingebrethsen and E. Matijetic, "Preparation of Uniform Colloidal Dispersions by Chemical Reactions in Aerosols2. Spherical Particles of Aluminum Hydrous Oxide," J. Aerosol Sci., 11 (2) (1980), pp. 271-280.
31. J.R. Carruthers et al., "Nonstoichiometry and Crystal Growth of Lithium Niobate," J. Appl. Phys., 42 (5) (1971), pp. 1846-1851.
32. Y. Fukukawa et al., "Optical Damage Resistance and Crystal Quality of LiNbO3 Single Crystals with Various [Li]/[Nb] Ratios," J. Appl. Phys., 72 (8) (1992), pp. 3250-3254.
33. K. Nassau and M. E. Lines, "Stacking Fault Model for Stoichiometry Deviations in LiNbO3 and LiTaO3 and the Effect on the Curie Temperature," J. Appl. Phys., 41 (2) (1970), pp. 533-537.
34. D.K. Fork, F. Armani-Leplingard, and J.J. Kingston, "Optical Losses in Ferroelectric Oxide Thin Films: Is There Light at the End of the Tunnel?," Mat. Res. Soc. Symp. Proc., vol. 361 (Pittsburgh, PA: MRS, 1995), pp. 155-166.
35. D.C. Bradley, R.C. Mehrotra, and. D.P. Gaur, Metal Alkoxides (London: Academic Press, 1978), p. 46.
36. K.W. Chour, G.D. Wang, and R. Xu, "Vapor Deposition of Lithium Tantalate with Volatile Double Alkoxide Precursors," Mat. Res. Soc. Symp. Proc., vol. 335 (Pittsburgh, PA: MRS, 1994), pp. 65-71.
37. K.W. Chour et al., "Autostoichiometric Vapor Deposition: Part III. A Study of Stoichiometry and Characterization of Epitaxial LiTaO3 Layer," J. Cryst. Growth, in press.
38. R.L. Barns and J.R. Carruthers, "Lithium Tantalate Single Crystal Stroichiometry," J. Appl. Cryst., 3 (1970), pp. 395-399.
39. S.C. Abrahams and J.L. Bernstein, "Ferroelectric Lithium Tantalate-1. Single Crystal X-Ray Diffraction Study at 24°C," J. Phys. Chem. Solids, 28 (1967), pp. 1685-1692.
ABOUT THE AUTHOR
Ren Xu received his Ph.D in materials science and engineering from the University of California, Los Angeles. He is currently an assistant professor of materials science and engineering at the University of Utah.
For more information, contact R. Xu, Department of Materials Science and Engineering, 304 EMRO, University of Utah, Salt Lake City, Utah 84112; (801) 581-6748; fax (801) 581-4816; e-mail REN.XU@MSE.UTAH.EDU.
Copyright held by The Minerals, Metals & Materials Society, 1997
Direct questions about this or any other JOM page to jom@tms.org.